Reflective Scientific Advisory Board: 2024 Meeting Report
Context:
Reflective’s mission is to equip the world with the data and tools needed to make informed decisions about SAI, fast enough to matter.
This requires building, maintaining and socializing a research roadmap, which outlines the critical path for research, including the dependencies and timelines, and which can be used to identify what is already being done well and where gaps remain.
Reflective’s Scientific Advisory Board (SAB) met in August 2024 to begin developing that roadmap: starting with identifying key uncertainties, determining what research or experiments will reduce them, and scoping out a rough sequence/schedule for priority areas. This report summarizes the key discussions and recommendations.
The discussion was bounded by Reflective’s research prioritization philosophy, which focused the discussion on:
- SAI, exclusively, at least for the time-being.
- Physical sciences and engineering, not social sciences, although we recognize the necessity of being aware of the broader context as it affects what research is needed, feasible, and how it is done.
- Knowledge critical to informing the nearest-term deployment options. We believe that “gen1” will be sulfate aerosols with gaseous precurors. There is more time to conduct research for further-out options.
A critical path towards which decision(s)?
Informed decisions about the deployment of SAI will require research addressing the following broad questions:
- What are the expected impacts of deployment? What are the impacts of SAI on people, ecosystems and economies? How would these impacts vary with different deployment scenarios? How would these impacts compare with no-SAI scenarios (risk-risk framework)?
- What is our level of confidence in these impacts? Which impacts are most uncertain? Which of these uncertainties are most consequential? What can be done to improve confidence? What scales/types of experiments are needed to reduce or resolve the most consequential uncertainties? What are the limits on what we can know?
- How might SAI be deployed? What are plausible deployment scenarios? What physical infrastructure and monitoring and attributional capabilities would be needed at experimental scale and deployment scale? How would one responsibly ramp up from the very start of deployment through to the latter stages of early deployment? What infrastructure is needed for the deployment process and how long will it take to build up?
The first two questions capture similar questions to what IPCC Working Group 1 would cover for climate change. The third has the potential to be more controversial. Nonetheless, we believe that the third question is important for four reasons:
- The impacts of SAI deployment are dependent on how SAI is deployed, so we cannot make claims about impacts without thoroughly considering the technical constraints and timelines posed by physical infrastructure and observational/attributional tools.
- The primary reason for conducting research into SAI is because it is conceivable that it will be deployed; as such it is important to conduct some of these long lead-time activities so that if there was a decision to deploy, the option would be available.
- Because a responsible SAI deployment would ramp up slowly, over decadal scales, some uncertainties may be reduced/resolved during early deployment, at scales too small to induce any associated climate impacts. As such, any assessment of uncertainty needs to take into account this potential for learning at small scales.
- This category of research is essential to inform governance and feasibility.
Mental models for research, outdoor experimentation and early deployment
We use the term “experiment” to refer to outdoor perturbative activities intended to reduce uncertainty in process representations. These are not at scales that would have any detectable surface climate response. |
Current research primarily involves modeling and observations of background conditions and natural analogs. However, these may be insufficient to inform a future decision about whether or not to deploy SAI, or to have the ability to respond wisely to deployment by a rogue actor. Eventually, controlled outdoor perturbative experiments will likely be needed to reduce/resolve the largest sources of uncertainty in relevant stratospheric processes and to validate model results.
In order to ensure that as much as possible can be learned from smaller-scale experiments, before potentially moving onto larger — and possibly riskier — experiments, we believe that there is a need/opportunity to sequence and stage-gate proposed SAI experiments. Stage-gating is common practice across many other fields. Clinical trials in medicine, for example, similarly aim to evaluate the safety and effectiveness of a new intervention in a carefully controlled, sequenced manner, and may serve as a helpful analogy. In clinical trials, a decision to progress to the next, larger “phase” of a clinical trial is only possible once pre-defined endpoints — outcome metrics about dosage, safety, or efficacy — have been met in the current phase.
In this vein, we do not believe that there will be a single go/no-go decision. Instead, we believe that there will be many points during the experimental phase to halt outdoor experiments. If there is a decision to deploy based on the full body of research, there will be continued learning — and multiple opportunities to continue, adjust, or stop deployment — during the decades of early deployment.
The figure below sketches out an illustrative research pathway. To note: while we’ve included illustrative numbers here, the specifics of these decision points are not for us to decide. Instead, we believe that this schematic provides a useful anchor for thinking about how to sequence research and potentially early deployment based on the scale and risk profile of these stages.
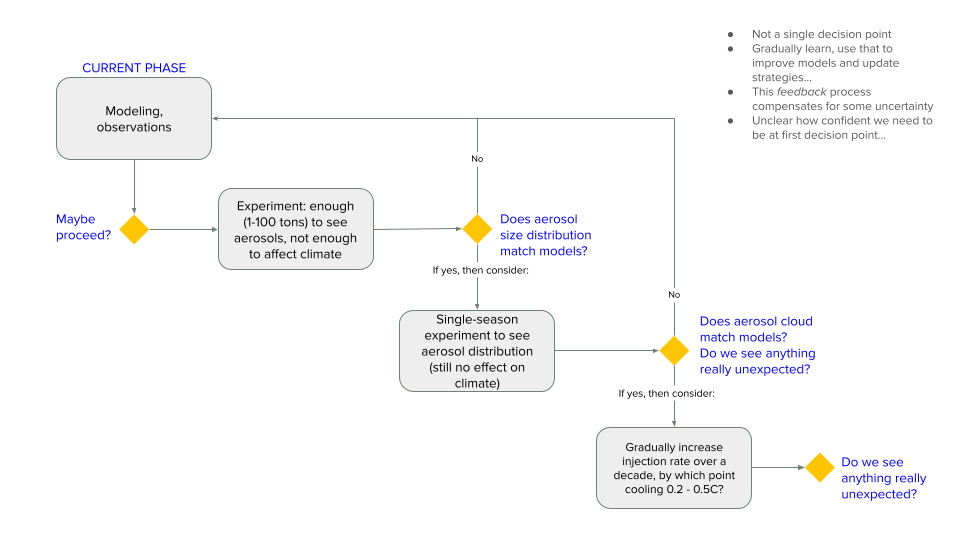
We are currently in the phase of “in silico” modeling and non-perturbative observations, the equivalent of the preclinical phase in medical clinical trials. This might be followed by an experiment on the scale of a single airplane flight, releasing between 1-100 tons of SO2. A potential subsequent phase would involve multiple airplane flights, but would still release far less material than what would affect the climate. One would only consider proceeding to early deployment if the results from these experiments were sufficiently consistent with predictions.
Furthermore, if a decision is made to deploy SAI, there are still decision points beyond that. We do not know how quickly future decision-makers may choose to ramp-up cooling. But with a current rate of warming ~0.23 °C per decade, it seems likely that it will take at least one decade, if not two, before we reach 0.5 °C of cooling.1 As such, this “early deployment” period offers an extended window to observe the climate response and validate model predictions. (Throughout, we use the term “early deployment” to refer to this period, starting from the point where there is an intent to detectably cool the planet and continuing to ramp up towards <0.5 °C of cooling.) Should observational data diverge from predictions or if detrimental effects were observed at any point during this period, policymakers would have the ability to continue to increase the amount of cooling, to hold the amount (or roughly, the injection rates) constant while continuing to monitor, or to phase out.
The main reason to choose the upper limit of “early deployment” as 0.5 °C is to provide a consistent baseline for assessing uncertainties and climate impacts; the specific value is less important than picking some value to enable consistent trade-offs. Since 0.5 °C would be at least a decade into deployment, this value would provide time to observe, learn from, and modify deployment. Additionally, the IPCC SR1.5 has described “robust” negative climatic and societal impacts with an increase in global mean temperatures from 1.5 °C to 2 °C above pre-industrial,2 and thus it is already well established that a change in global mean temperature of 0.5 °C is big enough to matter for impacts. (For a high-latitude deployment, there may be similar but more appropriate metrics to use than global mean temperature.)
This guides us to prioritize research to inform a future decision on whether to start early deployment of SAI — research that informs decisions to begin a gradual ramp up towards something less than 0.5 °C of cooling — and deprioritize research into impacts that are undetectable at this level. It also deprioritizes the question of how much cooling to ultimately do, if there’s a decision to move to full-scale deployment, until further data has been collected. Moreover, it means that if there is a risk of a deleterious climate impact at large-scale deployment, but we could study the related uncertainties at scales small enough where any negative impacts would still be minor, we’d have the choice to halt ramp-up based on new data, which limits the odds of experiencing this particular bad outcome. Failing to take this into account results in inappropriately assessing the range of possible outcomes from some uncertainty.
Uncertainty assessment
The first SAB meeting focused on identifying key uncertainties, assessing their magnitude and importance, and identifying what research, including potential experiments, could help reduce or resolve the most consequential ones. This does not capture all of the research questions that need to be answered, but we believe captures several critical path efforts that have not currently received sufficient attention. The SAB intentionally did not focus on uncertainties/activities that are covered well by others.
Process:
- The SAB created a long-list of uncertainties, without any effort to rank or filter. See Appendix A for a comparison of the SAB’s list of uncertainties to lists generated by other efforts.
- The SAB populated, based on the expert opinion of the SAB members, a two-by-two matrix. See Appendix B for a table with the rationale for the placement of each uncertainty from a subset of prioritized uncertainties.
- X-axis: how big is the uncertainty? How wrong could we be? How wide is the confidence interval?
- Y-axis: how consequential is the uncertainty? If we were wrong, how much would this affect our understanding of decision-relevant climate impacts/risks? What is our current guess about how this uncertainty would affect the ability to make future decisions?
- For a subset of highly consequential uncertainties, the group identified questions that should be answered:
- Does this uncertainty need to be resolved in the short/medium/long term?
- What can be done to reduce the uncertainty (or the influence of the uncertainty); can it be reduced through additional modeling, additional observations, or with perturbative experimentation? [Note: there are a number of uncertainties that we know a priori that outdoor experiments cannot resolve]
- If so, what’s the (observational or perturbative) experiment that would reduce/resolve the uncertainty? (what scale, what type) How much would the proposed experiment allow us to constrain? (i.e. would we be able to resolve entirely, reduce uncertainty by 20%, etc.)
- For perturbation experiments, what’s the risk of the proposed experiment?
- The SAB sketched out a rough sequencing for reducing/resolving high-consequence uncertainties, including any dependencies.
Three priorities for SAI research
The SAB meeting highlighted three priorities: reducing the uncertainty related to aerosol size distribution and small scale physics, time-critical questions about how to observe plumes and small-scale deployment, and long-lead time engineering questions for initial delivery systems.
The selection of our priority activities was also informed by an assessment of dependencies and timelines. Research activities that require urgent investment, either because they’re long lead-time activities or because significant downstream work depends on their timely completion, were prioritized.
Additionally, we selected priorities based on our understanding of where gaps exist in current funding and research priorities. In selecting these three to prioritize, we don’t mean to imply that there aren’t other critical research priorities as well, but rather that these three in particular are currently under-resourced.
1) Address the uncertainty relating to aerosol size distribution and small-scale physics.
Note: consistent with Reflective’s research prioritization philosophy, we are focusing on sulfate aerosols, released as a gaseous precursor.
Rationale: The Scientific Advisory Board identified aerosol size distribution as a priority, both because there is significant uncertainty about the ultimate aerosol size distribution, and potentially significant consequences if current estimates are wrong. Currently, most climate models used to study SAI have a horizontal resolution of, at best, 100 km and a vertical resolution in the stratosphere of, at best, 1 km. These “grid boxes” are far too large to resolve the turbulent mixing, aerosol chemistry, and microphysics that determine how a plume of SO2 released from an aircraft flying at stratospheric altitudes would evolve. It is instead common to simply increase the SO2 concentration in one or more grid boxes of these global models; this effectively assumes that the emitted SO2 has been uniformly mixed. Uncertainty over what happens at small scales results in uncertainty in the ultimate size distribution of aerosols, and hence both the efficiency (reflected sunlight for a given mass of sulfate) and the lifetime in the stratosphere.
Improving our understanding of aerosol microphysics, and then better representing this in our models could have huge consequences: at the moment, the amount of material that needs to be injected to achieve a given amount of cooling can vary by a factor of 2 or more. While this likely isn’t a show-stopper, better understanding aerosol formation, transport, and background composition, all of which influence the ultimate size distribution, — and potentially, learning what could be done to get closer to the theoretical ideal (where all sulfur particles are ½ a micron) — is critical for understanding how much needs to be injected, some of the potential impacts (e.g. acid rain), and the costs/feasibility of deployment. Moreover, this is critical for the overall legitimacy of SAI research: until we can state more confidently the amount that will need to be injected, it is hard to confidently state that we understand the effects of deployment.
An outdoor experiment to study aerosol microphysics (including effects from mixing and diffusion) is also likely to be the smallest scientifically-necessary test. A critical question to answer is how big this test would need to be; the amount of material to be injected needs to be estimated through a combination of modeling and experimental design, but might be roughly the amount of material that would be released from one modified plane, flying once (so no more than 10-100 tons). Experimental design for releasing and studying this small amount of material will be critical because it will be challenging to track the dispersion of such a small plume over the 2-4 week period over which aerosol formation occurs.
Note that observations after volcanic eruptions will also be invaluable. However, volcanic eruptions will never be a perfect analog for the conditions under SAI deployment, and thus these observations alone cannot fully resolve this uncertainty.
This workstream will concurrently develop the needed small-scale models that can represent aerosol microphysics, define observational requirements for a small-scale test, and figure out how the test could be run and observed.
Why now: Better understanding of aerosol behavior is a linchpin towards moving forward with informed decision-making. Many of these activities, including modeling, designing experiments, securing support for and ultimately executing small-scale experiments, and then using observational data to improve/validate models, have long lead times. Furthermore, understanding how one would track and monitor one airplane’s worth of SO2 release is a precursor to understanding any subsequent larger activities, including the first days and weeks of a potential deployment. It is thus a critical path topic that we need to start on now.
What experiments/research do we have in mind?
- Develop state-of-the-art, turbulence-resolving (down to sub-meter resolution) Large Eddy Simulations (LES) combined with aerosol microphysics to model, in detail, the chemistry/microphysical conditions in an aircraft plume in the stratosphere, the evolving conditions as the plume mixes and spreads, and the resulting implications for ultimate size distribution.
- Design of an outdoor experiment that will track and analyze the microphysics of an aerosol plume from release until most aerosols have formed 2-4 weeks later. It is critical to design this controlled experiment to match as closely as possible the conditions of an actual SAI deployment; this will be complementary to efforts to obtain better observational data for volcanic plumes.
- Use forecast models to determine how to constrain aerosol distribution from observations through combination of forecast models and defining what observations are needed.
- Perform (laboratory) flow tube experiments for aerosol coagulation kinetics and optical properties of aerosol modifications that might change size distribution.
- Use modeling and (when available) experimental data to improve the microphysical parameterization used in climate models.
2) Assess the feasibility and trade-offs associated with proposed early deployment scenarios, including research on the engineering feasibility of the physical deployment infrastructure.
Rationale: Most of the scenarios currently being modeled assume that we have the ability to deploy at >20km (ARISE-SAI, for example, injects at 21.6km at four latitudes: 15°S, 15°N, 30°S, 30°N). And these scenarios typically run for decades without changes to deployment strategy.
However, because it is unlikely that this is how deployment starts, these scenarios are limited in their ability to provide proper impact assessments. For example, early deployments could be done using existing aircraft (e.g. modified business-jets) deploying closer to the poles, where the tropopause is lower. But there is currently not enough research being performed on these high-latitude, low-altitude scenarios, and furthermore it remains unclear what is actually possible, and how long it would take to develop deployment capability.
In order to better model the impacts of early deployment, we believe it is critically important to better understand possible options for how deployment could start, based on engineering feasibility studies, supply chain analyses, and deployment operations research. This requires understanding the timelines and investment case that would be required for specialized aircraft development, the requirements, feasibility and costs to convert existing aircraft for use for (lower-altitude) polar deployment, and extensive work to understand the tradeoffs (in terms of efficiency, health impacts, and climatic effects) of deploying between 13-15 km. And it requires funding modeling studies of these short-term deployment scenarios to understand what could be learned over time, how that information could be used, and what observational assets would be required. (Note that this workstream overlaps with the next, defining observational needs.)
Why now: The feasibility of different deployment approaches will have huge implications for what scenarios are studied, what experiments are run, and what engineering activities are prioritized for the near future, and even for development of policy (e.g., what actors could deploy). While unlikely, one illustrative example: if developing specialized aircraft were proven to be the only possible route to deployment (e.g. if converting existing aircraft proved infeasible even for early deployment at high latitudes), that would suggest needing to consider how to radically accelerate aircraft R&D, even with current levels of uncertainty about if/how SAI will proceed.
What experiments/research do we have in mind?
- Airframe engineering and aircraft modification engineering assessments to inform options for early deployment scenarios (e.g. high-latitude, low-altitude injections).
- On-board storage tank and nozzle design for release of SO2 gas to ensure well-mixed release.
- Use above research to inform how deployment could start and model these scenarios, generating data on the risks and opportunities of each approach.
3) Define an observational plan for experiments and early deployment (from single-aircraft scale to 0.5°C cooling)
Rationale: Both for SAI research and for the ability to manage an SAI deployment, it is critical to be able to measure aerosol properties, their spatial distribution within the stratosphere, as well as stratospheric composition and chemistry. Yet a clear plan for exactly what needs to be measured and how is currently lacking. This plan needs to be articulated across a range of scales from the single-aircraft test described earlier, to initial year of deployment where the aerosols are spread more globally but still at low concentrations, through to scaled deployment; these scales may lead to different needs in coverage and resolution. Platforms for observations include satellite, aircraft, and balloon for a combination of remote and in situ observations. Ensuring these observations is thus one of the longest lead-time efforts, yet we don’t yet have a clear articulation of what to measure, how, and how that information can be used to determine large-scale impacts based on low-levels of injection and effectively manage deployment.
At the same time, we’re currently poised to lose a few of our most significant assets for stratospheric observation. This includes the Microwave Limb Sounder (MLS) launched by NASA in 2004 as part of the Aura mission, which will cease operations in 2026 and is currently the only instrument measuring stratospheric water vapor, transport tracers, and halogenated species with daily near-global coverage. There are instruments proposed for launch in the 2030s that will perform stratospheric observations, and the more equipped they are to handle the observational requirements for SAI, the better. If these instruments are not sufficient, it would be important to consider launching SAI specific instruments.
We believe it’s important to define the observational requirements to observe aerosol properties (size, radiative characteristics, spatial distribution) and chemistry at different scales, identify what capabilities would be needed, and to the extent possible support inclusion of these capabilities in upcoming instrument launches.
Why now: Given the timelines for development and launch of satellites and other observational assets, and the obsolescence of the existing Aura MLS instrument and others, there’s an almost certain 2026-2029 gap, even in the most rushed scenario, of a similar instrument. Similarly many airborne observations are being conducted with aircraft that are over 50 years old. Observational systems take time to develop, and those operational systems will need time to obtain the baseline observations that are a prerequisite to a responsible deployment. Therefore, it is essential to figure out how to use current ground-based as well as proposed and existing satellite instruments to best perform the necessary observations. It is equally important to determine observational requirements that are not covered by these instruments, and how to solve for this gap.
What experiments/research do we have in mind?
- Develop an operational analysis testbed to enable quantifiable assessments of the value of different observational assets (including satellite and in-situ).
- Define observational requirements for experimental through deployment scales. This involves first determining signal to noise on different variables at different release scales to assess what could conceivably be learned. Next, analysis of how perfect observational information could be used, and, finally, assessing what information would be sufficient.
- Analyze how those observational requirements could be met through a combination of satellite and in-situ observations. For example, this might include an evaluation of whether we need to replace MLS wholesale.
Other areas discussed
Below are other key areas that the SAB discussed that fall outside the scope of the discussion about specific uncertainties and research priorities.
What about a potential assessment?
While a comprehensive assessment is important, it is both too large and out of scope for Reflective. While Reflective could potentially contribute by proposing a “menu” of assessment options, we do not plan on performing an assessment ourselves.
How will we include Global South researchers?
It is important for Reflective to fund a diversity of researchers to reach our goals. Right now, much of the research on SAI in the Global South focuses on impacts. Since this is not our initial focus, we will work to include highly-qualified technical researchers on the listed buckets for underrepresented regions. Additionally, Reflective’s simulator serves as a first step in allowing those interested from around the world to see how SAI will impact their regions. The SAB also had a limited discussion of the possibility of funding data infrastructure projects that enable more participation from researchers in the Global South.
How will we decide what groups to fund and how will we inform their research process?
Reflective’s leadership team will decide what grant candidates to consider by utilizing an open call, invited list, or sole invitation depending on the research area. The SAB will advise Reflective on the research areas we prioritize, but will not make decisions on what specific groups to fund.
Appendix A – Comparison of our list of uncertainties with other efforts
There have been other efforts to create uncertainty assessments for SAI or more generally sunlight reflection, and there are inevitably some differences between ours and others. Here is a non-exhaustive list of other efforts: Geoengineering Modeling Research Consortium (GMRC), Kravitz and MacMartin (2020), National Academies of Sciences, Engineering, and Medicine (NASEM), White House Office of Science and Technology Policy (OSTP), SilverLining Research Roadmap for Scientific Assessment.
We have conducted some internal review of these efforts, and while they are largely overlapping, there are differences. First, we don’t list the aerosol type and precursor as a key uncertainty. This is consistent with Reflective’s research prioritization philosophy, which states that we are focusing on SAI using sulfur released as a gaseous precursor. Second, while there are many uncertainties listed elsewhere surrounding SAI governance that are important to consider, we do not include these as we are focused on the scientific uncertainties. Some of the previous exercises focus only on the first two categories of research questions that we listed at the beginning, and did not focus on the question of how one might deploy; this is one of the more significant departures relative to prior efforts as these uncertainties are essential to resolve, have long lead-times, and are currently under-resourced relative to other aspects of the research agenda. Finally, we have endeavored to pick a consistent baseline at which the countervailing risks from climate change are already known to be clear — we chose 0.5 °C of cooling. At this level of cooling, the SAB felt that some of the uncertainties included in other efforts would not be large enough to affect a decision to start “early deployment.”
Appendix B – Rationale for the placement of each uncertainty
Uncertainty | Rationale for placement |
Technical feasibility | In order to make decisions about whether or not to deploy, it is critical to understand what is possible, which can be broken down into two areas: 1) the nearer-term involving modifying existing aircraft for high-latitude, but lower-altitude (13-15 km) “polar” deployment3 and 2) better understanding timelines and feasibility for lower-latitude, higher-altitude (18-22 km) deployment.3 For the first, additional research is also needed into the polar injection strategy itself. Second, there are currently no planes with the ability to fly to the higher altitude needed for lower latitude injection, however engineering capabilities make this seem feasible with sufficient development time. Additionally, for both areas there is a necessity to understand how to retrofit planes with nozzles, tanks, and other parts required to release the actual material. Due to the time necessary and the critical nature of it for deployment, we consider this overall uncertainty of the highest importance. |
Observational requirements and plans | In order to prepare for potential deployment, it is essential that tools are in place to monitor and gather data on such a scenario. This requires 1) A plan for using observations during a deployment (or experiment) to validate models. 2) Determination of the needed observations. 3) Platforms and instrumentation to obtain these observations. These capabilities may include in-situ, LiDAR, and satellite measurements. The uncertainty surrounding the latter is compounded by the fact that two of the space instruments currently used to measure stratospheric composition — NASA’s Microwave-Limb Sounding (MLS) instrument and Canada’s ACE-FTS — are set to go offline in the next few years. |
Aerosol size distribution | This is one of the largest physical-process uncertainties surrounding SAI that could potentially be substantially reduced prior to a deployment decision. The uncertainty in aerosol size distribution arises from uncertain microphysics — particularly from small scale processes. However, current climate models used to simulate cooling scenarios have a resolution of, at best, 100 km horizontally and 1 km vertically. This is not nearly fine enough to explicitly simulate the aerosol formation in the plume one releases nor how the aerosol spreads. Additionally, questions surrounding size distribution also stem from uncertainties in how the material is injected. Its somewhat high consequence stems from the facts that aerosol size distribution will impact how much cooling will occur (or conversely, the injection rate needed for a given cooling). |
Observational baseline | This is of significant consequence because we need to know where we are starting from in order to analyze changes due to SAI in a potential early-deployment scenario. To make decisions on whether or not deployment is to continue, we need to be able to determine how much it has perturbed the atmosphere from pre-injection levels. Reducing the above uncertainty is a prerequisite to adequately address this. |
Stratospheric transport | There are uncertainties in the circulation patterns in the stratosphere that affect the spatial distribution of injected aerosols. Furthermore, stratospheric heating from the aerosols will change stratospheric circulation; the effect on aerosol spatial distribution may not be significant at low cooling (though the effect is nonlinear), but these changes also affect surface climate and high-latitude stratospheric ozone concentrations.4 Nailing down these changes in stratospheric transport is essential towards gaining a clearer picture of SAI-induced changes. |
Precision of feedback control | Feedback control is used in model simulations to determine the magnitude of injection required to reach a desired amount of cooling. While effective feedback algorithms have been developed for SAI in simulations,5 it remains unclear how this process would unfold in the real world, and hence the limitations. There are many other factors needing further investigation including additional degrees of freedom4 and the impacts of internal, unforced climate variability.6 |
Effect of aerosols on radiative forcing | While we understand that stratospheric aerosol will induce a negative radiative forcing, there are uncertainties on how accurately our physical understanding is coded in climate models. |
Stratospheric chemistry | This uncertainty focuses on how stratospheric aerosols will lead to changes in stratospheric chemistry, and in particular the effects on ozone. The processes are relatively well understood, largely centering around increased aerosol surface area density (SAD), changes in transport, and changes in stratospheric temperature. |
Climate response to stratospheric heating | It is widely agreed upon that the sulfate aerosols introduced by SAI cause stratospheric heating due to their partial absorption of incoming and outgoing radiation. It is less certain, however, the impact this has on the climate. The stratospheric heating could have impacts including decreased precipitation and changes in atmospheric dynamics — namely the Hadley Circulation and Quasi-Biennial Oscillation.7 The heating of the tropical tropopause/lower stratosphere would cause increases in stratospheric water vapor, counteracting some of the aerosol cooling.8 However, at 0.5 °C of cooling these changes are likely to be small, hence any uncertainty about the changes are less critical. |
Aerosol-cloud interactions | This uncertainty centers on how sulfur aerosols will impact high-level cirrus clouds. Cirrus clouds trap outgoing longwave radiation, therefore having a positive radiative forcing on the Earth. If there is a change in the thickness/amount of these clouds, it will change this forcing and in turn global mean temperature. There is high uncertainty surrounding this as different studies have displayed both positive and negative impacts on high cirrus clouds.9 That being said, this forcing — whether positive or negative — is shown to have a small magnitude of change relative to the direct forcing from the aerosols, and therefore a minor impact on overall radiative balance. |
Climate response to stratospheric aerosol forcing | In addition to uncertainties in the stratospheric distribution of aerosols resulting from a specified injection strategy, there is uncertainty in how the large-scale climate responds to the resulting (shortwave) aerosol forcing and associated impacts on temperature and precipitation. Some of these could in principle be compensated through strategies that limit large meridional temperature gradients, but it is unclear how feasible this is in practice. For example, northern hemisphere injection is needed to stabilize AMOC, southern hemisphere to maintain Antarctic ice shelves, and an appropriate balance between these to maintain ITCZ, and thus it remains unclear whether all three of these can simultaneously be maintained. This is a very large bucket of uncertainties, including not just the atmosphere (including cloud responses), but also the ocean and land surface responses. These can and need to be reduced through careful modeling, though they are not amenable to being reduced through testing (and indeed some uncertainty would persist well into any deployment). In the future this uncertainty will need to be disaggregated. |
Impacts (health, ecosystems, agriculture, etc.) | It is essential to consider the ultimate impacts associated with SAI (or with climate change) and how those are influenced both by changes in the climate, as well as other pathways (such as changes to ozone, UV, acid rain, direct/diffuse light ratio, etc). These include changes to ecosystems, agriculture, air quality, exposure to extremes, and many other areas. In many cases these impacts could be influenced through multiple pathways, with an uncertain net effect. While there is existing research ongoing in many of these areas, significant additional research will be required. In many cases this is similar to impact assessment research under climate change, in other cases additional research will be needed to address SAI-specific pathways through which impacts could be affected. While in some cases there is confidence that SAI could improve some impacts relative to no intervention — with the extent of improvement unclear — in other areas SAI adds to impacts (e.g., for acid rain). |
- Forster, P. M., Smith, C. J., Walsh, T., Lamb, W. F., Lamboll, R., Hauser, M., Ribes, A., Rosen, D., Gillett, N., Palmer, M. D., Rogelj, J., von Schuckmann, K., Seneviratne, S. I., Trewin, B., Zhang, X., Allen, M., Andrew, R., Birt, A., Borger, A.,…Zhai, P. (2023). Indicators of Global Climate Change 2022: annual update of large-scale indicators of the state of the climate system and human influence, Earth Syst. Sci. Data, 15, 2295–2327, https://doi.org/10.5194/essd-15-2295-2023 ↩︎
- IPCC, 2018: Headline Statements from the Summary for Policymakers. In: Global Warming of 1.5°C. An IPCC Special Report on the impacts of global warming of 1.5°C above pre-industrial levels and related global greenhouse gas emission pathways, in the context of strengthening the global response to the threat of climate change, sustainable development, and efforts to eradicate poverty [Masson-Delmotte, V., P. Zhai, H.-O. Pörtner, D. Roberts, J. Skea, P.R. Shukla, A. Pirani, W. Moufouma-Okia, C. Péan, R. Pidcock, S. Connors, J.B.R. Matthews, Y. Chen, X. Zhou, M.I. Gomis, E. Lonnoy, T. Maycock, M. Tignor, and T. Waterfield (eds.)]. Cambridge University Press, Cambridge, UK and New York, NY, USA, pp. 3-24, doi:10.1017/9781009157940.001 ↩︎
- Bednarz, E. M., Butler, A. H., Visioni, D., Zhang, Y., Kravitz, B., and MacMartin, D. G. (2023). Injection strategy – a driver of atmospheric circulation and ozone response to stratospheric aerosol geoengineering. Atmos. Chem. Phys., 23, 13665–13684, https://doi.org/10.5194/acp-23-13665-2023 ↩︎
- Bednarz, E. M., Visioni, D., Butler, A. H., Kravitz, B., MacMartin, D. G., & Tilmes, S. (2023). Potential non-linearities in the high latitude circulation and ozone response to stratospheric aerosol injection. Geophysical Research Letters, 50, e2023GL104726. https://doi.org/10.1029/2023GL104726 ↩︎
- Kravitz, B., MacMartin, D. G., Mills, M. J., Richter, J. H., Tilmes, S., Lamarque, J.-F., Tribbia, J.J., Vitt, F. (2017). First simulations of designing stratospheric sulfate aerosol geoengineering to meet multiple simultaneous climate objectives. Journal of Geophysical Research: Atmospheres, 122, 12,616–12,634. https://doi.org/10.1002/2017JD026874 ↩︎
- Connolly, C., Prewett, E., Barnes, E. A., & Hurrell, J. W. (2024). Quantifying the impact of internal variability on the CESM2 control algorithm for stratospheric aerosol injection. Earth’s Future, 12, e2023EF004300. https://doi.org/10.1029/2023EF004300 ↩︎
- Wells, A. F., Henry, M., Bednarz, E. M., MacMartin, D. G., Jones, A., Dalvi, M., & Haywood, J. M. (2024). Identifying climate impacts from different stratospheric aerosol injection strategies in UKESM1. Earth’s Future, 12, e2023EF004358. https://doi.org/10.1029/2023EF004358 ↩︎
- Bednarz, E. M., Visioni, D., Kravitz, B., Jones, A., Haywood, J. M., Richter, J., MacMartin, D. G., Braesicke, P. (2023). Climate response to off-equatorial stratospheric sulfur injections in three Earth system models – Part 2: Stratospheric and free-tropospheric response. Atmospheric Chemistry and Physics, 23, 687–709. https://doi.org/10.5194/acp-23-687-2023 ↩︎
- MacMartin, D.G., Kravitz, B., Long, J.C.S. and Rasch, P.J. (2016), Geoengineering with stratospheric aerosols: What do we not know after a decade of research?. Earth’s Future, 4: 543-548. https://doi.org/10.1002/2016EF000418 ↩︎